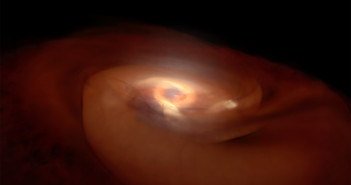
The Birth of Disks Around Protostars
The dusty disks around young stars make the news regularly due to their appeal as the birthplace of early exoplanets. But how do disks like these first form and evolve around their newly born protostars? New observations from the Atacama Large Millimeter/submillimeter Array (ALMA) are helping us to better understand this process.
Formation from Collapse
Stars are born from the gravitational collapse of a dense cloud of molecular gas. Long before they start fusing hydrogen at their centers — when they are still just hot overdensities in the process of contracting — we call them protostars. These low-mass cores are hidden at the hearts of the clouds of molecular gas from which they are born.
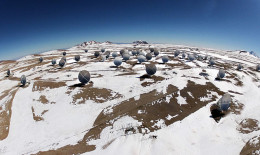
Aerial image of the Atacama Large Millimeter/submillimeter Array. [EFE/Ariel Marinkovic]
But how do these Keplerian disks — which eventually have scales of hundreds of AU — first form and grow around protostars? We need observations of these disks in their early stages of formation to understand their birth and evolution — a challenging prospect, given the obscuring molecular gas that hides them at these stages. ALMA, however, is up to the task: it can peer through to the center of the gas clouds to see the emission from protostellar cores and their surroundings.
![ALMA observations of the protostar Lupus 3 MMS. The molecular outflows from the protostar are shown in panel a. Panel b shows the continuum emission, which has a compact component that likely traces a disk surrounding the protostar. [Adapted from Yen et al. 2017]](https://aasnova.org/wp-content/uploads/2017/03/fig3-260x529.jpg)
ALMA observations of the protostar Lupus 3 MMS. The molecular outflows from the protostar are shown in panel a. Panel b shows the continuum emission, which has a compact component that likely traces a disk surrounding the protostar. [Adapted from Yen et al. 2017]
New Disks Revealed?
In a recent publication led by Hsi-Wei Yen (Academia Sinica Institute of Astronomy and Astrophysics, Taiwan), a team of scientists presents results from ALMA’s observations of three very early-stage protostars: Lupus 3 MMS, IRAS 15398–3559, and IRAS 15398–2429. ALMA’s spectacular resolution allowed Yen and collaborators to infer the presence of a 100-AU Keplerian disk around Lupus 3 MMS, and signatures of infall on scales of <30 AU around the other two sources.
The authors construct models of the sources and show that the observations are consistent with the presence of disks around all three sources: a 100-AU disk around a 0.3 solar-mass protostar in the Lupus system, a 20-AU disk around a 0.01 solar-mass protostar in IRAS 15398–3559, and 6-AU disk around a 0.03 solar-mass protostar in IRAS 15398–2429.
By comparing their observations to those of other early-stage protostars, the authors conclude that in the earliest protostar stage, known as the Class 0 stage, the protostar’s disk grows rapidly in radius. As the protostar ages and enters the Class I stage, the disk growth stagnates, changing only very slowly after this.
These observations mark an important step in our ability to study the gas motions on such small scales at early stages of stellar birth. Additional future studies will hopefully allow us to continue to build this picture!
Citation
Hsi-Wei Yen et al 2017 ApJ 834 178. doi:10.3847/1538-4357/834/2/178