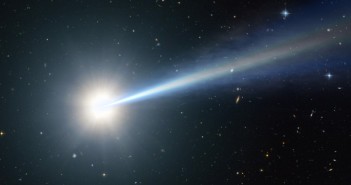
ALMA Examines a Distant Quasar Host
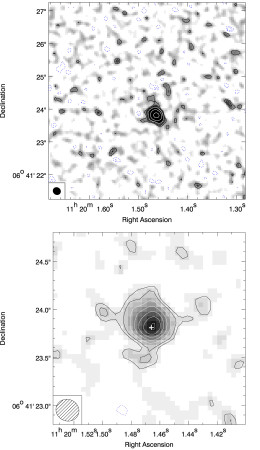
The dust continuum (top) and the [CII] emission (bottom) maps for the region around J1120+0641. [Adapted from Venemans et al. 2017]
Faraway Monsters and Their Galaxies
We know that quasars — the incredibly luminous and active centers of some distant galaxies — are powered by accreting, supermassive black holes. These monstrous powerhouses have been detected out to redshifts of z ~ 7, when the universe was younger than a billion years old.
Though we’ve observed over a hundred quasars at high redshift, we still don’t understand how these early supermassive black holes formed, or whether the black holes and the galaxies that host them co-evolved. In order to answer questions like these, however, we first need to gather information about the properties and behavior of various supermassive black holes and their host galaxies.
A team of scientists led by Bram Venemans (Max-Planck Institute for Astronomy, Germany) recently used the unprecedented sensitivity and angular resolution of ALMA — as well as the Very Large Array and the IRAM Plateau de Bure Interferometer — to examine the most distant quasar currently known, J1120+0641, located at a redshift of z = 7.1.
A High-Resolution Look
The team’s observations of the dust and gas emission from the quasar’s host galaxy revealed a number of intriguing things:
- The majority of the galaxy’s emission is very compact. Around 80% of the observed flux came from a region of only 1–1.5 kpc in diameter.
- Despite the fact that the 2.4-billion-solar-mass black hole at the galaxy’s center is accreting at a high rate, the heating in the galaxy is dominated not by the black hole’s accretion, but by star formation.
- There’s no sign of the expected structure of a rotating disk on kpc scales.
- The authors estimate a dynamical mass of the host galaxy of 43 billion solar masses — and the black hole at the galaxy’s center makes up ~6% of that. This ratio is roughly 10x higher than the black-hole-to-bulge mass ratio in local early-type galaxies.
- In the very central region, the black hole accounts for around 20% of the galaxy’s dynamical mass, and gas and dust likely accounts for most of the remainder. This doesn’t leave much room for massive stars in the center of the galaxy.
ALMA’s capabilities have enabled these first efforts to spatially resolve the host galaxy of the most distant quasar known, resulting new and unexpected information. The authors now look hopefully to the future, when even longer baselines of ALMA may allow us a still-higher-resolution look at this distant quasar, possibly providing answers to some of the questions it has raised.
Citation
Bram P. Venemans et al 2017 ApJ 837 146. doi:10.3847/1538-4357/aa62ac