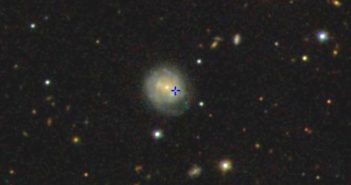
Unexpected Discovery of a Bright Cow
The recent discovery of an unusual transient event, nicknamed “the Cow”, has set the community of transient astronomers abuzz (amoo?). What do we know about this odd event so far?
Thinking Outside the Box
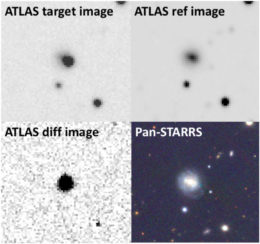
The location of AT2018cow: a post-discovery image (top left), a pre-discovery reference image (top right), a subtracted difference image (bottom left), and a Pan-STARRS multi-color image (bottom right). [Prentice et al. 2018]
Among the large variety of new classes of transients uncovered by these surveys are supernova-like events whose lightcurves rise and fall much faster than standard supernovae. One example is AT2017gfo, the first confirmed kilonova, which was paired with the neutron-star merger first detected in gravitational waves in August 2017. Additional examples of these rapidly evolving transients span a wide range of peak absolute magnitudes (from –15 to –22 magnitude) and rise times (~1–10 days), making them difficult to explain through a single scenario.
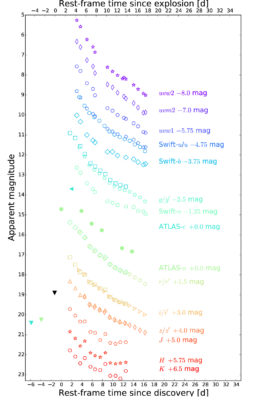
ATLAS, Liverpool Telescope, GROND, and Swift light curves of AT2018cow. [Adapted from Prentice et al. 2018]
An Unusual Transient
The Cow was first discovered with ATLAS, a twin 0.5-m telescope system located in Hawaii, on the night of 16 June 2018. Post-discovery monitoring of the Cow with various telescopes spanning optical, near-infrared, and ultraviolet wavelengths reveals a variety of odd properties.
The Cow’s peak luminosity was remarkably high: ~1.77 x 10^44 erg/s, or about 10–100 times brighter than a typical supernova. It reached the peak very quickly, brightening by more than 5 mag in just 3.3 days, while typical supernovae have rise times of perhaps 10–20 days. In addition, the Cow had a high peak blackbody temperature (~27,000 K), low estimated ejecta mass (just 0.1–0.4 solar mass), and relatively featureless and non-evolving spectra.
Magnetar from a Collision?
The combination of the Cow’s odd properties eliminates a number of more common progenitor explanations, such as supernova shock breakout. The authors do explore one scenario that could produce properties similar to the Cow’s, however: the formation of a magnetar — a strongly magnetized neutron star — from the merger of a binary neutron star system. Such a model, Prentice and collaborators say, would predict a transient with a peak luminosity, decline rate, and effective temperature that are all consistent with those of the Cow.How can we confirm this picture? The next step will be to compare additional observations of AT2018cow in radio and X-ray wavelengths — which were made simultaneously with those reported here in near-infared through ultraviolet — to the magnetar models to see if the models also match those observations. If so, we may have an explanation for this unusual transient.
Citation
“The Cow: Discovery of a Luminous, Hot, and Rapidly Evolving Transient,” S. J. Prentice et al 2018 ApJL 865 L3. doi:10.3847/2041-8213/aadd90