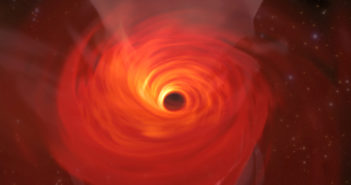
The Appearance of a Black Hole’s Shadow
In April of this year, the Event Horizon Telescope captured the first detailed images of the shadow of a black hole. In a new study, a team of scientists has now explored what determines the size and shape of black hole shadows like this one.
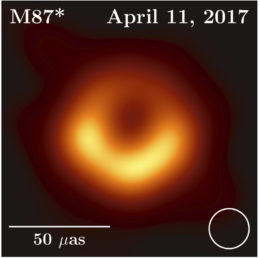
The first detailed image of a black hole, M87, taken with the Event Horizon Telescope. [Adapted from EHT collaboration et al 2019]
Imaging a Shadow
The stunning new radio images of the supermassive black hole in nearby galaxy Messier 87, released this spring by the Event Horizon Telescope team, revealed a bright ring of emission surrounding a dark, circular region.
This distinct structure is a result of the warped spacetime around massive objects like black holes. The ring of light is comprised of photons from the hot, radiating gas that surrounds the black hole, whose paths have been bent around the black hole before arriving at our telescopes. The dark region in the center is termed the black hole’s “shadow”; this is the collection of paths of photons that did not escape, but were instead captured by the black hole.
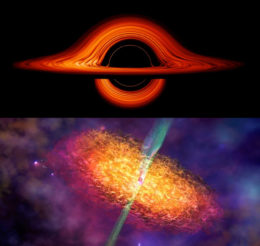
Comparison of conceptions of a black hole surrounded by a thin accretion disk vs. a thick accretion disk. [Top: NASA, bottom: Nicolle R. Fuller/NSF]
The Shape of Accretion
While some previous studies have explored what a black hole shadow looks like when the black hole is surrounded by a very thin disk of accreting gas (think the black hole + disk from the movie Interstellar), most supermassive black holes — like M87, or our own supermassive black hole, Sagittarius A* — are more likely to be surrounded by hot, accreting gas that is more broadly distributed, forming a thick or quasi-spherical disk.
Does the geometry and motion of the accreting gas affect the size and shape of a black hole’s shadow?
Models of Monsters
In a new study, three scientists — Ramesh Narayan and Michael Johnson (Harvard-Smithsonian Center for Astrophysics) and Charles Gammie (University of Illinois at Urbana–Champaign) — have teamed up to explore how a black hole’s shadow changes based on the behavior of the hot gas around it.
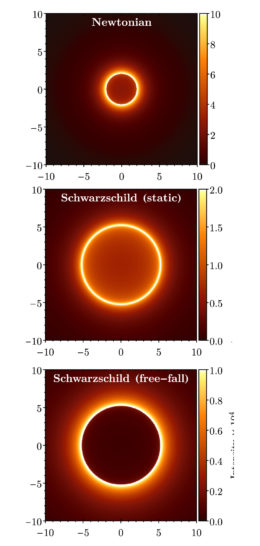
The image of the black hole shadow for three of the authors’ models: non-relativistic spacetime (top), relativistic spacetime with static surrounding gas (center), and relativistic spacetime with accreting gas flowing radially inwards (bottom). [Adapted from Narayan et al. 2019]
Reducing Complications
Intriguingly, the authors found that the appearance of the black hole’s shadow doesn’t depend on the details of the gas accretion close to the black hole. The size of the shadow was primarily determined by the spacetime itself (which is impacted by the mass of the black hole). But how the gas is distributed around the black hole, and whether that gas is stationary or accreting, doesn’t hugely affect the appearance of the shadow.
Real life is a little messier than this simple, spherically symmetric model; black hole spin and the presence of jets or outflows will cause asymmetries in the shadow. But the authors’ results generally tell us that the close-in details of accretion flows aren’t complicating what we’re seeing. And that’s valuable information we can use as we interpret future observations of black hole shadows!
Citation
“The Shadow of a Spherically Accreting Black Hole,” Ramesh Narayan et al 2019 ApJL 885 L33. doi:10.3847/2041-8213/ab518c