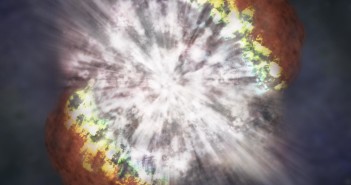
The Shape of Superluminous Supernovae
What causes the tremendous explosions of superluminous supernovae? New observations reveal the geometry of one such explosion, SN 2015bn, providing clues as to its source.
A New Class of Explosions
Supernovae are powerful explosions that can briefly outshine the galaxies that host them. There are several different classifications of supernovae, each with a different physical source — such as thermonuclear instability in a white dwarf, caused by accretion of too much mass, or the exhaustion of fuel in the core of a massive star, leading to the core’s collapse and expulsion of its outer layers.In recent years, however, we’ve detected another type of supernovae, referred to as “superluminous supernovae”. These particularly energetic explosions last longer — months instead of weeks — and are brighter at their peaks than normal supernovae by factors of tens to hundreds.
The physical cause of these unusual explosions is still a topic of debate. Recently, however, a team of scientists led by Cosimo Inserra (Queens University Belfast) has obtained new observations of a superluminous supernova that might help address this question.
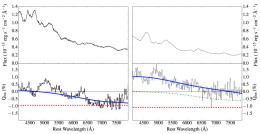
The flux and the polarization level (black lines) along the dominant axis of SN 2015bn, 24 days before peak flux (left) and 28 days after peak flux (right). Blue lines show the author’s best-fitting model. [Inserra et al. 2016]
Probing Geometry
Inserra and collaborators obtained two sets of observations of SN 2015bn — one roughly a month before and one a month after the superluminous supernova’s peak brightness — using a spectrograph on the Very Large Telescope in Chile. These observations mark the first spectropolarimetric data for a superluminous supernova.
Spectropolarimetry is the practice of obtaining information about the polarization of radiation from an object’s spectrum. Polarization carries information about broken spatial symmetries in the object: only if the object is perfectly symmetric can it emit an unpolarized spectrum. Otherwise, the polarization of an object’s spectrum reveals information about its geometry.
Modeling Ejecta
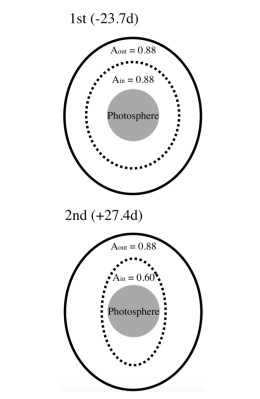
The authors’ best model of the geometry of SN 2015bn 24 days before (top) and 28 days after (bottom) peak flux. The model consists of two ellipsoidal layers of ejecta material. [Inserra et al. 2016]
To explain these dependencies, the authors produce a simple toy model of SN 2015bn. In the best-fitting model, the supernova has a two-layered ellipsoidal or bipolar geometry. The inner region becomes more and more aspherical as time passes.
What does this model tell us about the physical cause of this superluminous supernova? Inserra and collaborators argue that the axisymmetric shape favors a core-collapse explosion. A central inner engine of a spinning magnetar (a highly magnetized neutron star) or black hole then remains at the center of this explosion, pumping energy into it and causing the increase of the inner asymmetry over time.
The authors caution that their models are very preliminary — but these observations should drive future, more detailed modeling, as well as further spectropolarimetric observations of future nearby superluminous supernovae. With luck, we will soon better understand what drives these unusual explosions.
Citation
C. Inserra et al 2016 ApJ 831 79. doi:10.3847/0004-637X/831/1/79