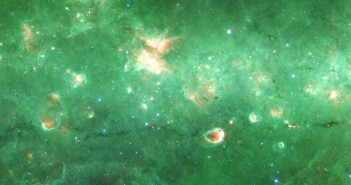
Companions for “Nessie” in the Milky Way’s Skeleton
The recent discovery of a purported “bone” of the Milky Way, a dark cloud nicknamed “Nessie”, has provided us with new clues for mapping out the spiral structure of our galaxy. It turns out that Nessie may not be alone: a follow-up study has identified more bones, potentially making up a skeleton of the Milky Way that traces out the densest parts of its spiral arms.
Inconvenient Vantage Point
How many spiral arms does the Milky Way have? Where are they located? What does the structure look like between the arms? It may seem surprising that these fundamental questions don’t yet have clear answers. But because we’re stuck in the galaxy’s disk, we’re forced to piece together our understanding of the Milky Way’s structure based primarily on measurements of position and radial velocity of structures within the galactic plane.
The discovery of Nessie presents an intriguing new tool to identify the layout of the galaxy. Nessie is a very long, thin, infrared-dark filament that runs along the modeled position of the Scutum-Centaurus arm — and is believed therefore to trace the structure of the arm. In a new study led by Catherine Zucker (University of Virginia, Harvard-Smithsonian Center for Astrophysics), the authors have searched for additional bones like Nessie, hoping to use them to map out the skeleton of the Milky Way.
New Bones Discovered
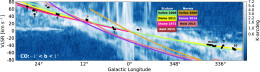
In this map of radial velocity vs. galactic longitude, the bone candidates are indicated by the numbered points. The colored lines indicate the positions of two of the galactic spiral arms, according to various models. Click for a closer look! [Zucker et al. 2015]
Next, the team obtained radial-velocity data for the candidates from five separate radio surveys. Five of the candidates did not have radial velocities consistent with the galactic rotation curve at the predicted positions of the nearby arms.
Hope for a Skeleton
The authors used the remaining ten candidates to construct rough criteria for an object to be a Milky Way “bone”:
- Largely continuous mid-infrared extinction feature
- Parallel to the galactic plane, to within 30°
- Position within 20 pc of the galactic mid-plane
- Radial velocity within 10 km/s of the predicted velocity of a Milky Way arm
- No abrupt shifts in velocity within the extinction feature
- Projected aspect ratio of ≥50:1
Of the ten candidates, six meet all the criteria and are thought to mark the location of significant spiral features in the galaxy. The authors believe that this method may be used to identify hundreds of Milky Way bones in the future. Combining this skeleton with other tracers of galactic structure will ultimately help us to piece together a more accurate map of our galaxy.
Bonus
Check out this video, produced by the authors using World Wide Telescope, that shows the locations of the newly discovered bone candidates within Spitzer images of the Milky Way galactic plane. [Credit: Zucker et al. 2015]
Citation
Catherine Zucker et al 2015 ApJ 815 23. doi:10.1088/0004-637X/815/1/23