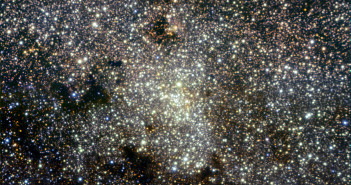
Metallicity of the Stars at the Galactic Center
A recent study suggests that the stars in the central parsec of our galaxy are not a single, roughly solar-metallicity population, as previously thought. Instead, these stars have a large variation in metallicities — which has interesting implications for the formation history of the Milky Way’s nuclear star cluster.
Clues from Abundances
Why do we care about the metallicity of stars and stellar populations? Metallicity measurements can help us to separate multiple populations of stars and figure out when and where they were formed.
Measurements of the chemical abundances of stars in the Milky Way have demonstrated that there’s a metallicity gradient in the galaxy: on average, it’s below solar metallicity at the outer edges of the disk and increases to above solar metallicity within the central 5 kpc of the galaxy.
So far, measurements of stars in the very center of the galaxy are consistent with this galactic trend: they’re all slightly above solar metallicity, with little variation between them. But these measurements exist for only about a dozen stars within the central 10 pc of the galaxy! Due to the high stellar density in this region, a larger sample is needed to get a complete picture of the abundances — and that’s what this study set out to find.
Different Populations
Led by Tuan Do (Dunlap Fellow at the University of Toronto and member of the Galactic Center Group at UCLA), the authors of this study determined the metallicities of 83 late-type giant stars within the central parsec of the galaxy. The metallicities were found by fitting the stars’ K-band spectra from observations by the NIFS instrument on the Gemini North telescope.
In contrast to the previous studies, the authors found that the 83 stars exhibited a wide range of metallicities, from a tenth of solar metallicity all the way to super-solar metallicities.
The abundances of the low-metallicity stars they found are consistent with globular cluster metallicities, suggesting that these stars (about 6% of the sample) may have arrived in the nuclear star cluster as a result of the infall of globular clusters. The super-solar metallicity stars were likely formed closer to the galactic center or from the disk.
The authors point out that current models of the star formation history and initial mass function of the nuclear stellar cluster — which typically assume a uniform population of stars with roughly solar metallicity — may need to be revisited in light of these results.
Citation
Tuan Do et al. 2015 ApJ 809 143 doi:10.1088/0004-637X/809/2/143