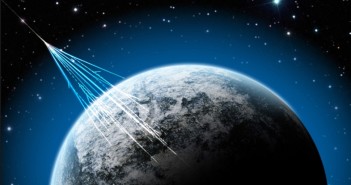
IceCube’s Search for Neutrinos from Gamma-Ray Bursts
In a cubic kilometer of volume of ice under Antarctica, an observatory called IceCube is taking measurements that may help us to determine what causes the ultra-high-energy cosmic rays (UHECRs) we occasionally observe from Earth. A recent study reports on its latest results.
Atomic Baseballs
Cosmic rays are high-energy radiation primarily composed of protons and atomic nuclei. When these charged and extremely energetic particles impact the Earth’s atmosphere on their journey through space, they generate showers of secondary particles that we then detect.
A UHECR is any cosmic-ray particle with a kinetic energy exceeding 1018 eV — and some have been detected with energies of more than 1020 eV! In practical terms, this is an atomic nucleus with the same kinetic energy as a baseball pitched at 60mph. These unbelievably energetic particles are quite rare, but we’ve observed them for decades. Yet in spite of this, the source of UHECRs is unknown.
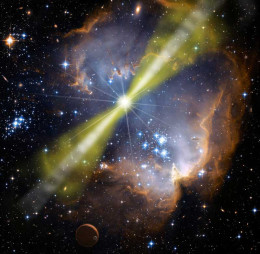
Illustration of a gamma-ray burst in a star-forming region. Could these phenomena accelerate UHECRs to their enormous energies? [NASA/Swift/Mary Pat Hrybyk-Keith and John Jones]
Gamma-Ray Burst Fireballs
One proposed source that could accelerate particles to these energies is a gamma-ray burst (GRB). In some models for GRBs, the explosion is envisioned as a relativistically expanding fireball of electrons, photons and protons. Internal shock fronts accelerate electrons and protons within the fireball, generating UHECRs, gamma rays, and neutrinos in the process.
Because the charged cosmic-ray particles can be easily deflected as they travel, it’s difficult to identify where they came from. Neutrinos and photons, on the other hand, both travel largely undeflected through the universe. As a result, if we detect high-energy neutrinos that are correlated with gamma-ray photons from a GRB, this would provide strong support for GRB fireball models for UHECR production.
Heading Under the Ice
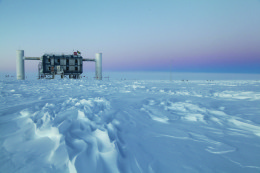
The IceCube Laboratory in Antarctica. Beneath the Antarctic ice lie more than 5,000 detectors over a cubic kilometer of volume. [IceCube/NSF/S. Lidstrom]
In a recently published study by the IceCube Collaboration, the team performed a three-year search for neutrinos that were correlated with the locations and times of more than 800 known GRBs during that period.
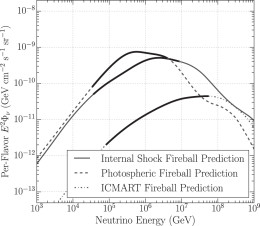
Three different fireball models for GRBs, and the predicted neutrino flux from each. The neutrinos potentially detectable by IceCube are shown with solid segments. IceCube’s detections (and lack thereof) place new constraints on these models. [Aartsen et al. 2016]
New Constraints
From three years of data, the collaboration reports the detection of five low-significance events correlated with five GRBs. But these events are also consistent with the background of charged particles generated in Earth’s atmosphere. What does this mean? These detections could indicate a small number of real neutrinos generated by GRBs — or they could just be background noise.
Either way, these results from IceCube provide a new upper limit on the association of neutrinos with gamma-ray bursts. This constrains which production mechanisms are possible, eliminating some models for UHECR acceleration by GRB fireballs.
What’s next? The collaboration indicates that the next generation IceCube-Gen2 detector, planned for the future, will be even more sensitive — which will either result in the detection of more subtle neutrino events associated with GRBs, or it will further disfavor GRBs as the production mechanism for UHECRs.
Citation
M. G. Aartsen et al 2016 ApJ 824 115. doi:10.3847/0004-637X/824/2/115