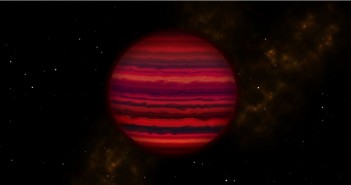
Water Clouds in the Atmosphere of a Jupiter-Like Brown Dwarf
Lying a mere 7.2 light-years away, WISE 0855 is the nearest known planetary-mass object. This brown dwarf, a failed star just slightly more massive than Jupiter, is also the coldest known compact body outside of our solar system — and new observations have now provided us with a first look at its atmosphere.
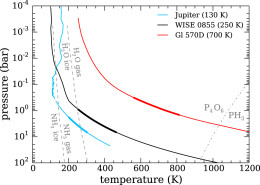
Temperature–pressure profiles of Jupiter, WISE 0855, and what was previously the coldest extrasolar object with a 5-μm spectrum, Gl 570D. Thicker lines show the location of each object’s 5-μm photospheres. WISE 0855’s and Jupiter’s photospheres are near the point where water starts to condense out into clouds (dashed line). [Skemer et al. 2016]
Challenging Observations
With a chilly temperature of 250 K, the brown dwarf WISE 0855 is the closest thing we’ve been able to observe to a body resembling Jupiter’s ~130 K. WISE 0855 therefore presents an intriguing opportunity to directly study the atmosphere of an object whose physical characteristics are similar to our own gas giants.
But studying the atmospheric characteristics of such a body is tricky. WISE 0855 is too cold and faint to be able to obtain traditional optical or near-infrared (< 2.5 µm) spectroscopy of it. Luckily, like Jupiter, the opacity of its gas allows thermal emission from its deep atmosphere to escape through an atmospheric window around ~5 µm.
A team of scientists led by Andrew Skemer (UC Santa Cruz) set out to observe WISE 0855 in this window with the Gemini-North telescope and the Gemini Near-Infrared Spectrograph. Though WISE 0855 is five times fainter than the faintest object previously detected with ground-based 5-µm spectroscopy, the dry air of Mauna Kea (and a lot of patience!) allowed the team to obtain unprecedented spectra of this object.
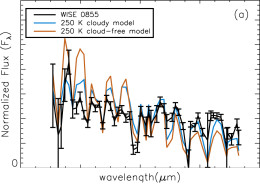
WISE 0855’s spectrum shows absorption features consistent with water vapor, and it’s best fit by a cloudy brown-dwarf model. [Skemer et al. 2016]
Water Clouds Found
Exoplanets and brown dwarfs cooler than ~350 K are expected to form water ice clouds in upper atmosphere — and these clouds should be thick enough to alter the emergent spectrum that we observe. Does WISE 0855 fit this picture?
Yes! By modeling the spectrum of WISE 0855, Skemer and collaborators demonstrate that it’s completely dominated by water absorption lines. This represents the first evidence of water clouds in a body outside of our solar system.
Atmospheric Turbulence
WISE 0855’s water absorption profile bears a striking resemblance to Jupiter’s. Where the spectra differ, however, is in the lower-wavelength end of observations: Jupiter also shows absorption by a molecule called phosphine, whereas WISE 0855 doesn’t.
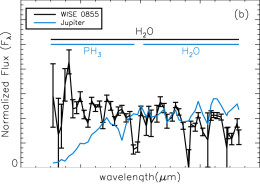
Jupiter’s spectrum is strikingly similar to WISE 0855’s from 4.8 to 5.2 μm, where both objects are dominated by water absorption. But from 4.5 to 4.8 μm, Jupiter’s spectrum is dominated by phosphine absorption, indicating a turbulent atmosphere, while WISE 0855’s is not. [Skemer et al. 2016]
These observations represent an important step as we attempt to understand the atmospheres of extrasolar bodies that are similar to our own gas-giant planets. Observations of other such bodies in the future — especially using new technology like the James Webb Space Telescope — will allow us to learn more about the dynamical and chemical processes that occur in cold atmospheres.
Citation
Andrew J. Skemer et al 2016 ApJ 826 L17. doi:10.3847/2041-8205/826/2/L17