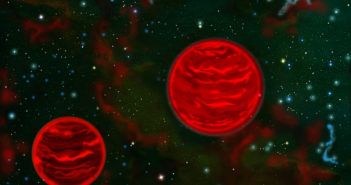
Discovery of a Free-Floating Double Planet?
An object previously identified as a free-floating, large Jupiter analog turns out to be two objects — each with the mass of a few Jupiters. This system is the lowest-mass binary we’ve ever discovered.
Tracking Down Ages
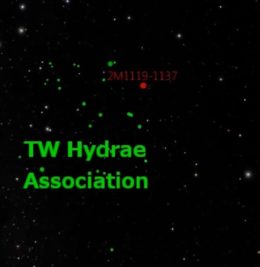
2MASS J11193254–1137466 is thought to be a member of the TW Hydrae Association, a group of roughly two dozen young stars moving together in the solar neighborhood. [University of Western Ontario/Carnegie Institution of Washington DTM/David Rodriguez]
This is surprisingly difficult, however. Brown dwarfs cool continuously as they age, which creates an observational degeneracy: dwarfs of different masses and ages can have the same luminosity, making it difficult to infer their physical properties from observations.
We can solve this problem with an independent measurement of the dwarfs’ masses. One approach is to find brown dwarfs that are members of nearby stellar associations called “moving groups”. The stars within the association share the same approximate age, so a brown dwarf’s age can be estimated based on the easier-to-identify ages of other stars in the group.
An Unusual Binary
Recently, a team of scientists led by William Best (Institute for Astronomy, University of Hawaii) were following up on such an object: the extremely red, low-gravity L7 dwarf 2MASS J11193254–1137466, possibly a member of the TW Hydrae Association. With the help of the powerful adaptive optics on the Keck II telescope in Hawaii, however, the team discovered that this Jupiter-like object was hiding something: it’s actually two objects of equal flux orbiting each other.
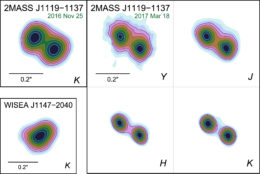
Keck images of 2MASS J11193254–1137466 reveal that this object is actually a binary system. A similar image of another dwarf, WISEA J1147-2040, is shown at bottom left for contrast: this one does not show signs of being a binary at this resolution. [Best et al. 2017]
Under this assumption, the authors then used the distance to the group — around 160 light-years — to estimate that the binary’s separation is ~3.9 AU. The assumed membership in the TW Hydrae Association also provides binary’s age: roughly 10 million years. This allowed Best and collaborators to estimate the masses and effective temperatures of the components from luminosities and evolutionary models.
Planetary-Mass Objects
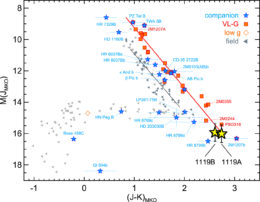
The positions of 2MASS J11193254–1137466A and B on a color-magnitude diagram for ultracool dwarfs. The binary components lie among the faintest and reddest planetary-mass L dwarfs. [Best et al. 2017]
Regardless of its definition, 2MASS J11193254–1137466AB qualifies as the lowest-mass binary discovered to date. The individual masses of the components also place them among the lowest-mass free-floating brown dwarfs known. This system will therefore be a crucial benchmark for tests of evolutionary and atmospheric models for low-mass stars in the future.
Citation
William M. J. Best et al 2017 ApJL 843 L4. doi:10.3847/2041-8213/aa76df