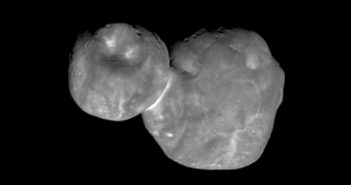
Insights from MU69’s (Lack of) Craters
One of the joys of getting new data from astronomy missions is the opportunity to test predictions. NASA’s New Horizons space probe is now beaming us data from its flyby of 2014 MU69 — and there’s a lot to learn!
A Distant Target
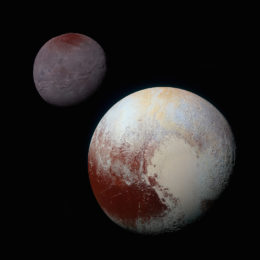
This composite image with enhanced colors shows New Horizons observations of Pluto (foreground) and Charon (background). These Kuiper-belt bodies have very few craters … will MU69 be similar? [NASA/JHUAPL/SwRI]
On 1 January 2019, New Horizons flew by MU69, passing within 3,500 km — three times closer than the probe approached Pluto! Now, the spacecraft is slowly sending us its stored, high-resolution images, and we’re getting a progressively more detailed look at this odd object.
What predictions can we hope to test as data continues to trickle in from New Horizons? One intriguing question was asked long before we had any views of this body: How cratered is MU69’s surface?
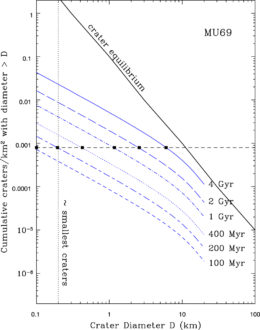
Authors’ predicted crater density above a given crater diameter vs. the crater diameter, with curves showing different times. The horizontal dashed line corresponds to 1 crater/MU69 surface. The vertical dotted line marks the smallest craters we’ll be able to detect with New Horizons images. These results suggest that after 4 billion years, only a few dozen craters of varying sizes will have formed. [Greenstreet et al. 2019]
What’s the Damage?
Months ago, a team of scientists led by Sarah Greenstreet (B612 Asteroid Institute and University of Washington) conducted a study in which they made predictions for the crater count they expected to find on MU69’s surface. Greenstreet and collaborators used observations of Pluto and Charon’s surfaces and models of known Kuiper-belt populations to explore the bombardment of MU69 over the solar system’s life span and calculate the number of craters of different sizes its surface should host.
The authors’ results were intriguing: they found that, despite getting bombarded for 4+ billion years, MU69 should be marred by very few craters. Greenstreet and collaborators estimate that MU69 should have only ~25–50 craters larger than ~200 m in size, which is the smallest size we’re likely be able to see with the full-resolution New Horizons images.
Awaiting Confirmation
Since the publication of Greenstreet and collaborators’ paper, we now have the first images of MU69 to compare to — and, as you can see for yourself in the cover image, its surface seems remarkably smooth. We’ll have to wait for the high-resolution images to finish downlinking to be sure (higher-resolution images are expected near the end of this month, and the full data are expected by September 2020), but so far, our views of MU69 seem to support the authors’ predictions.
What implications would this confirmation have? First, this would mean that MU69 is a sample of the primordial solar system: it now much like it was when it first formed ~4 billion years ago. This is in stark contrast to, for instance, the main-belt asteroids, which have undergone considerable evolution since they formed.
In addition, MU69’s low crater count may imply that there are far fewer small bodies in the Kuiper belt than we had originally expected. This scarcity would have far-reaching implications for what solar-system-formation models are possible, potentially changing our understanding of how our planets were built.Keep sending along your data, New Horizons! We can’t wait to see what we’ll learn.
Citation
“Crater Density Predictions for New Horizons Flyby Target 2014 MU69,” Sarah Greenstreet et al 2019 ApJL 872 L5. doi:10.3847/2041-8213/ab01db