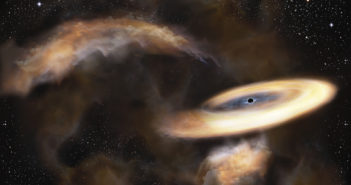
The Contradiction of a Low-Mass Massive Black Hole
The black holes we’ve observed in the universe typically fall into two categories: small star-sized black holes, and gargantuan black holes lurking at the centers of galaxies. Now, a new black-hole discovery sheds some light on the gray area between these extremes.
Growing Together
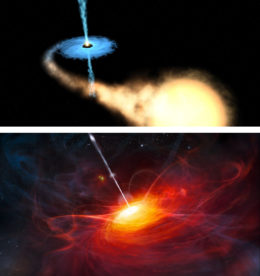
Illustrations of two types of accreting black holes: a stellar-mass black hole accreting from a binary companion (top) and a supermassive black hole accreting gas in a galaxy’s center (bottom). [Top: ESA/NASA/Felix Mirabel; Bottom: ESO/M. Kornmesser]
Intriguingly, the mass of these central black holes seems to be inherently tied to that of their host. An empirical relationship known as the M-σ relation shows a correlation between a central black hole’s mass and the spread of star velocities in its host galaxy’s bulge, which acts as a proxy for the bulge mass. The M-σ relation and other, similar relationships show that black holes seem to grow in tandem with their host galaxies throughout the universe.
If the M-σ relation holds across a broad range of masses, then we would expect to find smaller massive black holes at the hearts of especially low-mass galaxies. So far, evidence for these low-mass central black holes has been scarce. But a new study led by Ingyin Zaw (New York University Abu Dhabi, UAE) has now delivered a low-mass massive black hole for us to contemplate.
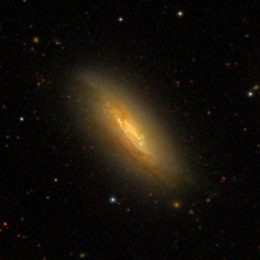
A 4’ x 4’ view of IC 750, a low-mass galaxy that hosts a massive (though less so than expected!) black hole at its center. [Sloan Digital Sky Survey]
Mass Measurement from Masers
Zaw and collaborators used the Very Long Baseline Array to obtain radio observations of the low-mass galaxy IC 750.
At the galaxy’s heart, the authors found emission from water masers, clumps of water molecules that emit light naturally in a process similar to laser emission. Light from the masers shows that they are orbiting in a disk around a compact central mass — a massive black hole — and Zaw and collaborators used their motion to measure the mass enclosed in their orbit, providing an upper limit on the black hole’s mass.
The authors then reduced and analyzed publicly available multiwavelength data to understand the location of the black hole and measure the properties of its host galaxy.
A Decidedly Low-Mass Monster
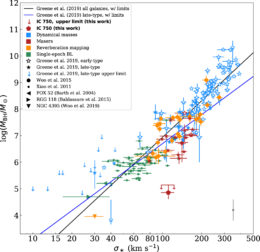
This plot of black hole mass vs. bulge stellar velocity dispersion (the M-σ relation) shows IC 750 marked with a red star, with upper mass limits indicated by downward-pointing arrows. It falls two orders of magnitude below where we’d expect it to lie on the relation. Click to enlarge. [Zaw et al. 2020]
What’s going on with this unusual object? There are two possible explanations: either there’s more scatter at the low-mass end of the M-σ relation, or the scaling relationship is simply different for low-mass galaxies. The latter option is supported by some simulations that suggest that black holes don’t grow efficiently in low-mass galaxies.
Though we don’t yet know which explanation is more likely, more observations like those presented here will eventually fill in our picture of these low-mass massive monsters.
Citation
“An Accreting, Anomalously Low-mass Black Hole at the Center of Low-mass Galaxy IC 750,” Ingyin Zaw et al 2020 ApJ 897 111. doi:10.3847/1538-4357/ab9944