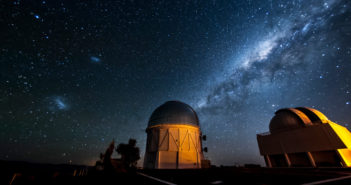
Supernovae, Dark Energy, and the Fate of Our Universe
What’s the eventual fate of our universe? Is spacetime destined to continue to expand forever? Will it fly apart, tearing even atoms into bits? Or will it crunch back in on itself? New results from Dark Energy Survey supernovae address these and other questions.
Uncertain Expansion
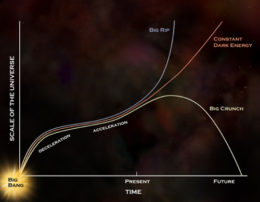
The evolution of the scale of our universe; click to enlarge. Measurements suggest that the universe is currently expanding, but does dark energy behaves like a cosmological constant, resulting in continued accelerating expansion like now? Or might we instead be headed for a Big Rip or Big Crunch? [NASA/CXC/M. Weiss]
Since this idea was first proposed, we’ve conducted decades of research to better understand what dark energy is, how much of it there is, and how it influences our universe.
In particular, dark energy’s still-uncertain equation of state determines the universe’s ultimate fate. If the density of dark energy is constant in time, our universe will continue its current accelerating expansion indefinitely. If the density increases in time, the universe will end in the Big Rip — space will expand at an ever-increasing acceleration rate until even atoms fly apart. And if the density decreases in time, the universe will recollapse in the Big Crunch, ending effectively in a reverse Big Bang.
Which of these scenarios is correct? We’re not sure yet. But there’s a project dedicated to finding out: the Dark Energy Survey (DES).
The Hunt for Supernovae
DES was conducted with the Dark Energy Camera at the Cerro Tololo Inter-American Observatory in Chile. After six years taking data, the survey officially wrapped up observations this past January.
One of DES’s several missions was to make detailed measurements of thousands of supernovae. Type Ia supernovae explode with a prescribed absolute brightness, allowing us to determine their distance from observations. DES’s precise measurements of Type Ia supernovae allow us to calculate the expansion of the space between us and the supernovae, probing the properties of dark energy.
Though DES scientists are still in the process of analyzing the tens of terabytes of data generated by the project, they recently released results from the first three years of data — including the first DES cosmology results based on supernovae.
Refined Measurements
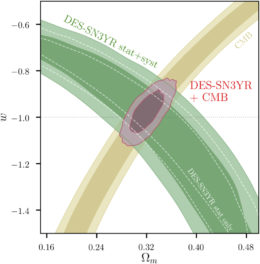
Constraints on the dark energy equation of state w from the DES supernova survey. Combining this data with constraints from the cosmic microwave background radiation suggest an equation of state consistent with a constant density of dark energy (w = –1). [Abbott et al. 2019]
From their observations, the DES team is also able to provide an estimate for the dark-energy equation of state w, finding that w = –0.978 ± 0.059. This result is consistent with a constant density of dark energy (w = –1), which would mean that our universe will continue to expand with its current acceleration indefinitely.
These results are exciting, but they use only ~10% of the supernovae DES discovered over the span of its 5-year survey. This means that we can expect even further refinements to these measurements in the future, as the DES collaboration analyzes the remaining data!
Citation
“First Cosmology Results using Type Ia Supernovae from the Dark Energy Survey: Constraints on Cosmological Parameters,” T. M. C. Abbott et al 2019 ApJL 872 L30. doi:10.3847/2041-8213/ab04fa