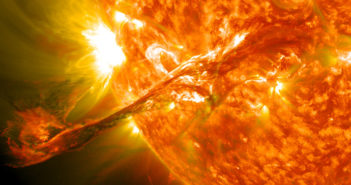
Which of Kepler’s Stars Flare?
The habitability of distant exoplanets is dependent upon many factors — one of which is the activity of their host stars. To learn about which stars are most likely to flare, a recent study examines tens of thousands of stellar flares observed by Kepler.
Need for a Broader Sample
Most of our understanding of what causes a star to flare is based on observations of the only star near enough to examine in detail — the Sun. But in learning from a sample size of one, a challenge arises: we must determine which conclusions are unique to the Sun (or Sun-like stars), and which apply to other stellar types as well.Based on observations and modeling, astronomers think that stellar flares result from the reconnection of magnetic field lines in a star’s outer atmosphere, the corona. The magnetic activity is thought to be driven by a dynamo caused by motions in the star’s convective zone.
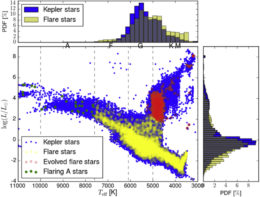
HR diagram of the Kepler stars, with flaring main-sequence (yellow), giant (red) and A-star (green) stars in the authors’ sample indicated. [Van Doorsselaere et al. 2017]
Intriguing Trends
Van Doorsselaere and collaborators used a new automated flare detection and characterization algorithm to search through the raw light curves from Quarter 15 of the Kepler mission, building a sample of 16,850 flares on 6,662 stars. They then used these to study the dependence of the flare occurrence rate, duration, energy, and amplitude on the stellar spectral type and rotation period.
This large statistical study led the authors to several interesting conclusions, including:
- Roughly 3.5% of Kepler stars in this sample are flaring stars.
- 24 new A stars are found to show flaring activity. This is interesting because A stars aren’t thought to have an outer convective zone, which should prevent a magnetic dynamo from operating. Yet these flaring-star detections add to the body of evidence that at least some A stars do show magnetic activity.
- Most flaring stars in the sample are main-sequence stars, but 653 giants were found to have flaring activity. As with A stars, it’s unexpected that giant stars would have strong magnetic fields — their increase in size and gradual spin-down over time should result in weakening of the surface fields. Nevertheless, it seems that the flare incidence of giant stars is similar to that of F or G main-sequence stars.
- All stellar types appear to have a small fraction of “flare stars” — stars with an especially high rate of flare occurrence.
- Rapidly rotating stars are more likely to flare, tend to flare more often, and tend to have stronger flares than slowly rotating stars.
As a next step, the authors plan to apply their flare detection algorithm to the larger sample of all Kepler data. In the meantime, this study has both deepened a few mysteries and moved us a step closer in our understanding of which stars flare — and why.
Citation
Tom Van Doorsselaere et al 2017 ApJS 232 26. doi:10.3847/1538-4365/aa8f9a