In 2015, the first detection of gravitational waves changed astronomy forever, opening an entirely new window onto the physics of extreme objects like black holes and neutron stars. Today, we’ll take a look at three research articles that predict new gravitational wave sources, probe the prospects of detecting gravitational waves from ultra-light black holes, and compare measurements of the low-frequency gravitational wave background with predictions from the population of supermassive black holes in the local universe.
Disks of Collapsing Stars
So far, the LIGO, Virgo, and Kagra (LVK) detectors have spotted gravitational waves from a bevvy of inspiraling objects: pairs of black holes, pairs of neutron stars, and black hole–neutron star duos. Using vast collections of quickly spinning neutron stars called pulsars, researchers have even found evidence for the muddled hum of distant supermassive black hole binaries.
Recently, Ore Gottlieb (Flatiron Institute and Columbia University) and collaborators explored the possibility of detecting gravitational waves from an entirely different type of source: the accretion disk surrounding a black hole born from the collapse of a rapidly spinning massive star (i.e., a collapsar).
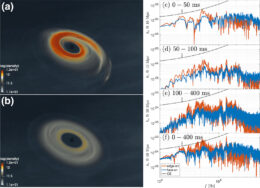
The accretion disk at early times, showing prominent spiral arms (panel a), and at later times when the disk has homogenized (panel b). The remaining panels show the predicted gravitational wave amplitude at different times and at different disk angles. Click to enlarge. [Gottlieb et al. 2024]
Gottlieb’s team estimates that the gravitational waves from the accretion disk of a collapsing star 33 million light-years (10 megaparsecs) away could be detected with a signal-to-noise ratio of dozens by current instruments. Potential future instruments like Cosmic Explorer would see the event more clearly, with a signal-to-noise ratio of hundreds. What these estimates translate to is a potential detection of dozens to hundreds of collapsars each year by future observatories — and the possibility that several of these events are already hidden somewhere in the existing LVK data, awaiting the analysis that would extract their convoluted signals from the noise.
Overall, Gottlieb and coauthors show that collapsars are a promising but as-yet-undetected source of gravitational waves. Be on the lookout for future research from these authors examining the specifics of gravitational wave emission from collapsar disks.
Black Holes in the Mass Gap
Until recently, observations suggested that black holes could be no lighter than 5 solar masses. In addition, neutron stars are expected to top out out somewhere between 2 and 3 solar masses. This leaves a void between 2–3 and 5 solar masses called the mass gap. The reasons for this gap are unclear, and new observations have begun to populate the gap with candidate objects, suggesting that the “gap” may not be a gap at all. If these detections are confirmed, researchers must figure out how these mass-gap objects came to be.
In a recent article, Claire Ye (University of Toronto) and collaborators explored how black holes in the mass gap might form, leading to a prediction of how often we should expect to detect these black holes in gravitational wave observations. The team considered three main formation pathways, all of which involve a neutron star that is too massive to support itself, causing it to collapse into a black hole:
- a neutron star forms via the collapse of a massive star’s core and accretes stellar material that falls back on to the neutron star
- a neutron star forms through the collision of two white dwarfs or the accretion of material onto a white dwarf
- a neutron star gains mass through collisions or tidal interactions with another star
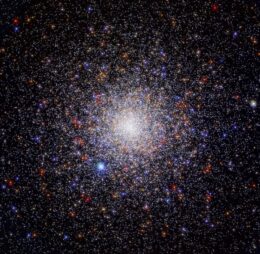
The densely packed globular cluster NGC 1851, as seen by the Hubble Space Telescope. [NASA, ESA, and G. Piotto (Università degli Studi di Padova); Processing: Gladys Kober (NASA/Catholic University of America)]
The simulations show that mass-gap black holes can be produced in dense star clusters, with different simulation runs producing anywhere from 4 to 465 mass-gap black holes. The main formation pathway also varied between simulations, with merging neutron stars or white dwarfs generally being the most common, except in more massive clusters, where core-collapse supernovae produced nearly as many mass-gap black holes as mergers did.
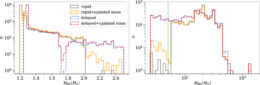
Neutron stars (left) and black holes (right) produced in globular clusters. The dividing line between neutron stars and black holes was set to be 2.5 solar masses. “Rapid” and “delayed” refer to the timescales over which supernova explosions play out. This factor has a large impact on the formation of mass-gap black holes. Click to enlarge. [Ye et al. 2024]
Bringing the Background to the Foreground
The last of today’s research articles concerns supermassive black holes in the nearby universe. The slow rumblings of countless supermassive black hole binaries are thought to generate a subtle background hum of gravitational waves at nanohertz frequencies. In 2023, research collaborations across the globe announced the discovery of significant evidence for the presence of the nanohertz gravitational wave background, creating new opportunities to test our understanding of the population of supermassive black holes in the universe.
Cataloging the masses of supermassive black holes at different points in cosmic history is critical for testing models of galaxy formation and black hole growth. The masses and numbers of supermassive black holes can also be used to predict the strength of the nanohertz gravitational wave background; comparing this prediction against observations can help test whether the census of black holes is complete, especially at the high-mass end that is thought to dominate the gravitational wave signal.
To study the highest-mass black holes, Emily Liepold and Chung-Pei Ma (University of California, Berkeley) undertook a study of the most massive galaxies in the nearby universe. The team used the results of the MASSIVE survey, which produced images and spectra of nearby massive galaxies. Leveraging existing dynamical and stellar-population modeling of a subset of these galaxies, they calculated the stellar mass of each galaxy in the MASSIVE sample. Then, using established relations between a galaxy’s stellar mass and the mass of its central black hole — the more massive the galaxy, the more massive the black hole — they assigned a black hole mass to each galaxy in their sample.
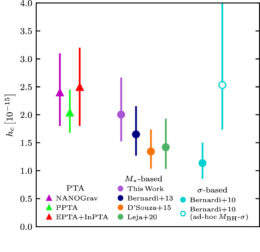
Comparison of the characteristic gravitational wave strain, or amplitude, calculated in this work (purple circle) to values calculated by other teams (circles of other colors) and observed using pulsar timing arrays (triangles). [Adapted from Liepold & Ma 2024]
Citation
“In LIGO’s Sight? Vigorous Coherent Gravitational Waves from Cooled Collapsar Disks,” Ore Gottlieb et al 2024 ApJL 972 L4. doi:10.3847/2041-8213/ad697c
“Lower-Mass-Gap Black Holes in Dense Star Clusters,” Claire S. Ye et al 2024 ApJ 975 77. doi:10.3847/1538-4357/ad76a0
“Big Galaxies and Big Black Holes: The Massive Ends of the Local Stellar and Black Hole Mass Functions and the Implications for Nanohertz Gravitational Waves,” Emily R. Liepold and Chung-Pei Ma 2024 ApJL 971 L29. doi:10.3847/2041-8213/ad66b8